The most fundamental function of nerve cells is the integration of their synaptic inputs to generate their
propagating output signal, the action potential. The major aims of Dr Nusser’s laboratory are to understand how
identified presynaptic nerve cells release neurotransmitters; how the released transmitter molecules activate
their postsynaptic receptors; and how the generated postsynaptic potentials are integrated to generate an action
potential. The Laboratory of Cellular Neurophysiology focuses on four major project areas using a variety of
molecular, neuroanatomical, in vitro electrophysiological, imaging and in silico modeling approaches:
1. Understand the role of identified nerve cells in olfaction. Pharmaco- and opto-genetic approaches are used
to modify the activity of nerve cells of the olfactory pathway while the animals perform odor discrimination
tasks.
2. Reveal the molecular, structural and functional heterogeneity of cortical excitatory and inhibitory synapses.
Determine the molecular specializations underlying the functional and structural diversity of synapses, such
as the probability and short-term plasticity of transmitter release, and the extent of postsynaptic receptor
activation. In vitro electrophysiology, two-photon imaging, light- and electron microscopic immunolocalization
are combined to address these issues.
3. Create a molecular map of the neuronal surface by determining the location and density of various voltageand ligand-gated ion channel subunits in defined subcellular compartments of identified nerve cells, using
quantitative light- and electron microscopic immunolocalization. Perform multi-compartmental modeling to
generate functionally testable prediction of the functional consequences of specialized ion channel
distributions. In vitro electrophysiology and imaging approaches are used to test the functional predictions of
our models.
4. Provide a quantitative description of the microcircuit of the cerebellar cortex with special focus on electrically
coupled GABAergic interneurons and their roles in network operations.
1. Understand the role of identified nerve cells in olfaction
The extraordinary diversity of nerve cells was already recognized over a century ago. It is now widely accepted that within most brain regions,
including the main olfactory bulb (MOB), glutamatergic principal cells are rather homogeneous, whereas GABAergic interneurons (INs) form a diverse cell population. The laboratory of Cellular Neurophysiology has identified
novel GABAergic IN subtypes in the MOB, which showed unique connectivity patterns. An interesting issue regarding the diversity of INs is identifying the role individual cell types might play in olfaction. Dr Nusser’s laboratory aims
to reveal the role of distinct cell types of the MOB and other olfactory brain areas using pharmaco- and opto-genetic approaches. Currently, three different pharmaco-genetic methods are used in the laboratory: 1) a method to employ zolpidem to selectively potentiate GABAA receptor-mediated
synaptic currents in a specific subset of neurons (Wulff et al., 2007, Nat Neurosci), 2) designer receptors engineered from human M3 muscarinic receptors mutated to be exclusively activated by the drug clozapine-N-oxide (CNO; Alexander et al., 2009, Neuron), 3) a mutated nAChR and GlyR chimera, which is selectively activated by a designer drug (Magnus et al.,2011, Science). These designer receptors and the wild-type GABAAR2 subunit will be delivered to the brain with stereotaxic injection of adenoassociated viruses either in a cell type-specific or a non-specific manner. Two to four weeks after the injections, the animals will be trained in odor discrimination tasks either in a freely moving or in a head-restrained way. The effects of pharmacological silencing of certain neuronal populations will be tested on the behavioral performance of the animals. In the head-restrained behavioral task, the cells will be also silenced by the use of light-activated archaerhodopsin.

2. Understanding the functional consequences of the
molecular and ultrastructural diversity of GABAergic and
glutamatergic cortical synapses
Understanding chemical synaptic neurotransmission in the CNS has
been in the spotlight of neuroscience for many decades. A tremendous
amount of information has been gathered regarding the molecular
events leading to the release of neurotransmitter from synaptic vesicles,
the diffusion of neurotransmitter molecules to their postsynaptic
receptors and the activation of these receptors. At the same time,
ultrastructural analysis of synapses revealed an enormous diversity in
the shape and size of pre- and postsynaptic structures among central
synapses. Recently, molecular approaches also revealed a large
number of molecules involved in pre- and postsynaptic function, and the
molecular diversity on many key players. However, it is still unknown
how functional heterogeneity of synapses is generated and how
alterations in synaptic geometry and molecular content affect synaptic
function. The Laboratory of Cellular Neurophysiology combines in vitro
electrophysiology and two-photon Ca2+ imaging with electron
microscopic analysis of hippocampal GABAergic and glutamatergic
synapses to address how diversity in the release probability and shortterm plasticity of release from distinct synapses correlate with the size
and shape of the presynaptic active zones. Furthermore, the laboratory
also employs quantitative electron microscopic freeze-fracture replica
immunogold localizations to reveal the molecular composition of
structurally and functionally distinct active zones. The results shed new
light on the structure-function relationship of central synapses and
provide a molecular explanation of why a larger active zone confers a
higher release probability at hippocampal CA3 pyramidal cell synapses.
These results also test the hypothesis that the ultrastructural differences
among synapses that belong to the same population (e.g. hippocampal
CA3 pyramidal cell to CA1 pyramidal cell connections) are the reflection
of molecular specializations that confer distinct functional properties.
Another area of interest in the laboratory is to gain an understanding of
the precise mechanism(s) of regulation of neurotransmitter release by
presynaptic neuromodulators. New combined imaging,
electrophysiological and molecular neuroanatomical approaches are
used to quantitatively characterize the presynaptic receptor content of
axon terminals that have been functionally imaged in acute slices.
Another aim is to determine how the molecular identity, number and
density of postsynaptic neurotransmitter receptors (e.g. AMPA, NMDA,
GABAA
) affect postsynaptic receptor occupancy and open probability at
identified hippocampal synapses.

3. Creating a molecular map of the neuronal surface
The most fundamental function of nerve cells is the integration of their synaptic
inputs to generate action potentials (APs). It is a generally accepted view that
APs are generated in the axon initial segment (AIS). However, input synapses
are usually distributed over a large dendritic tree. Because of this spatial
arrangement, the distance between a synapse and the site of output generation
varies to a tremendous extent, resulting in differential filtering of postsynaptic
responses by the dendrites. Thus, if dendrites were passive, the effect of a
synapse on output generation would depend on its dendritic location. However,
in the past decade, it has become apparent that dendrites of most nerve cells are
not passive, but contain a large number of voltage-dependent conductances,
which endow dendritic trees with an unanticipated computational power. The
molecular identity, exact location and density of voltage-gated ion channels in
small subcellular compartments on the axo-somato-dendritic surface determine
their roles in synaptic integration and output generation.
Many investigations focusing on the distribution of voltage-gated ion channels in
central neurons reached generalized conclusions such as ‘the voltage-gated K+
channel subunit Kv1.1 is axonal’ or ‘the HCN1 subunit is somato-dendritic’. Our
work using high resolution immunolocalization clearly demonstrated that many
ion channel subunits show different cell surface distribution patterns in distinct
neuron types (cell type-specific distributions). The fact that the ‘axonal’ and
‘somato-dendritic’ domains contain many functionally relevant compartments
introduces an additional level of complexity. The ‘axonal domain’ can be divided
into an AIS, nodes of Ranvier, myelinated axon segments, preterminal nonmyelinated axon segments, axon terminals and presynaptic AZs - compartments
that have specific functional roles that require different ion channels (and many
other molecules). Our recent results lead to another important conclusion; the
ion channel content of distinct subcellular compartments is highly specific
(subcellular compartment-specific distributions).
The laboratory of Dr Nusser has studied the subcellular distribution of a variety of
voltage-gated ion channel subunits (HCN1, Kv4.2, Kv1.1, Kv2.1, Nav1.6) using
light- and electron microscopic immunohistochemical approaches and reached
the conclusion that, so far, all examined ion channel subunits have different axosomato-dendritic distributions on the surface of hippocampal CA1 pyramidal
cells. In future experiments, the laboratory aims to extend these investigations to
other voltage-gated ion channels. In addition, in silico multi-compartmental
modeling is being used to create functionally testable predictions of the functional
consequences of different ion channel distributions. In vitro patch-clamp
electrophysiology and two-photon imaging will be carried out to test the model
predictions.

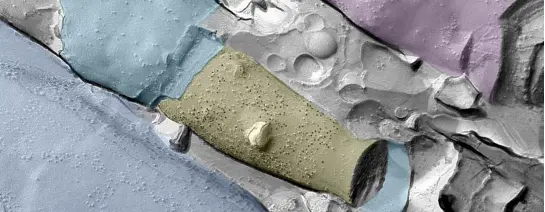